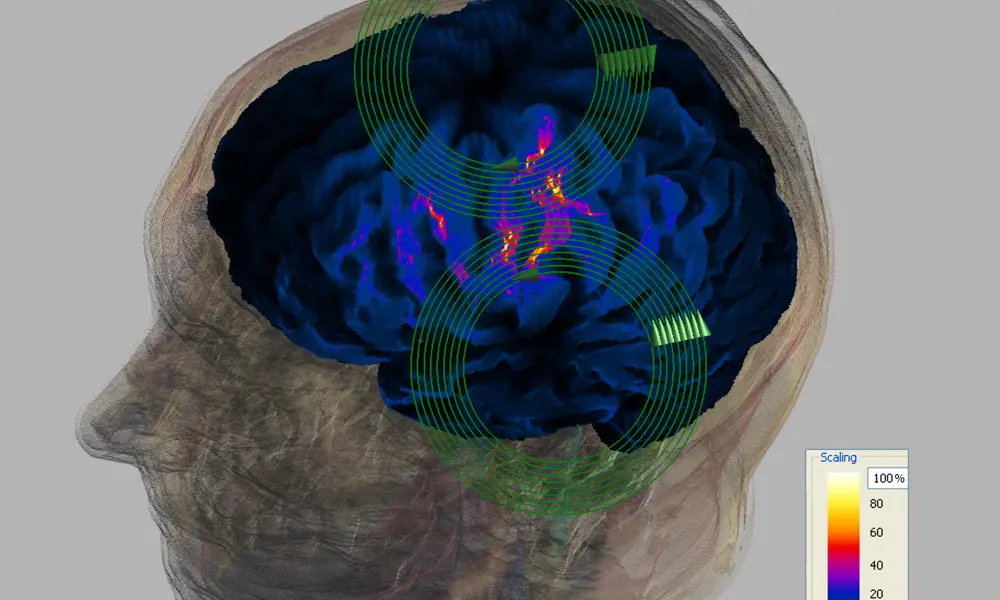
Transcranial magnetic stimulation
The development of non-invasive technologies has fundamentally changed the practice of modern medicine. From electrocardiograms to nuclear magnetic resonance imaging, clinicians are acquiring an ever more complete understanding of what is happening inside the body without surgery having to open it, providing a significant aid in diagnosing a variety of diseases.
Therapies have also benefited from the advent of the non-invasive revolution. Externally applied energy in the form of ultrasound, electric or magnetic fields and various forms of radiation have all given rise to non-invasive methods of treatment. Now, magnetic stimulation of nerves and the brain is coming into clinical practice, having been used for years as a research tool. Used initially to diagnose and monitor of the progress of some neurological diseases, it has now found a role in treatment, notably of depression and migraine.
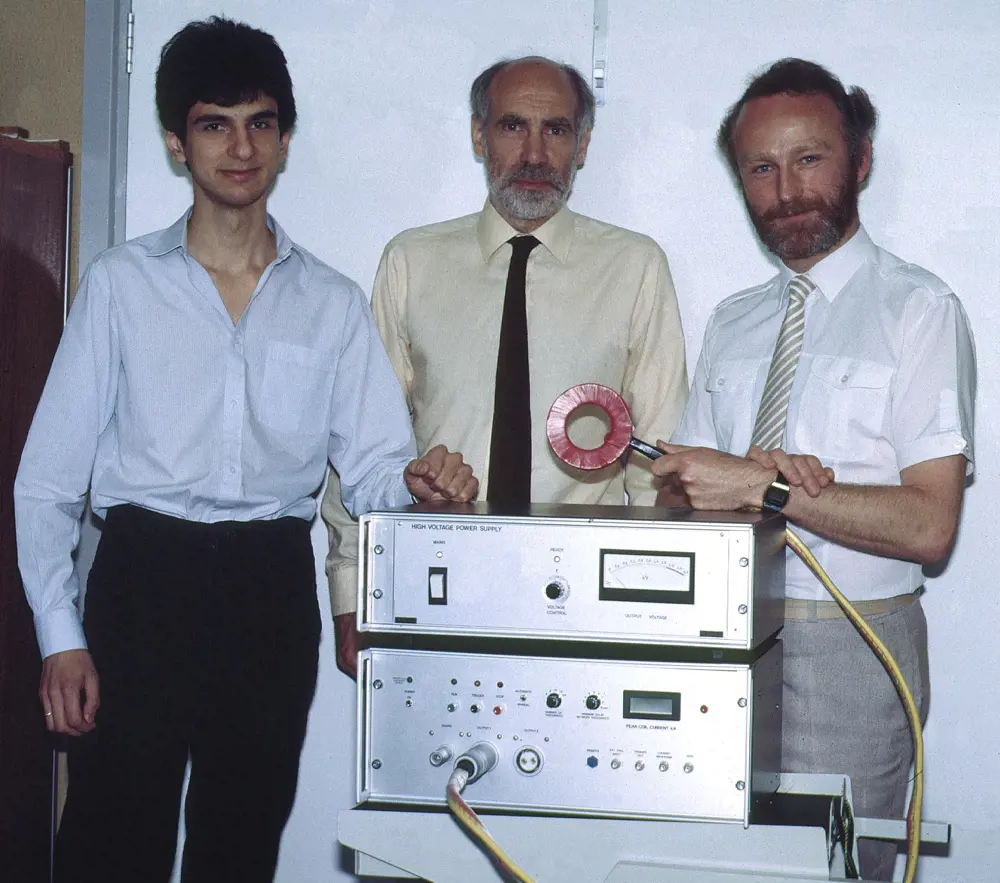
The first stimulator with which TMS was demonstrated in February 1985. Left to right – Dr Reza Jalinous, Professor Ian Freeston and Professor Tony Barker
Slow development
Observations on the interaction of electricity and living tissue go back to the experiments of Luigi Galvani in the late 1700s. He discovered that electrical stimulation of nerves using a rudimentary battery formed by a zinc and copper rod could cause muscles to contract. In the following century, Michael Faraday discovered that a time-varying magnetic field could generate electricity in conducting material – so called ‘electromagnetic induction’. However, it was not until the 1980s that researchers were able to develop technologies to allow these phenomena to be combined into the technique of magnetic stimulation, used to stimulate nerves and the human brain for therapy.
The reasons for this slow progress are a matter of speculation, but technical and cultural factors may both have played a part. Culturally, the standing of electricity and magnetism in therapy may have been constrained by the legacy of exaggerated and misguided Victorian beliefs in its alleged powers.
More prosaically, magnetic stimulation also had to wait for solutions to the practical engineering problems of developing the right lab equipment, and the vision to apply it to modern medical use.
Nerves act as an information conduit and carry electrical signals around the body to enable many of its functions. In the 1970s, an NHS and Sheffield University engineer, Anthony Barker, was studying the range of speeds at which large nerve fibres conduct signals in a nerve bundle (typically 40–80 m/s), in the hope of developing a diagnostic tool for diseases such as multiple sclerosis in which nerve conduction velocity is impaired. This required the nerve in question to be artificially stimulated with an externally applied electrical current. The problem was that human tissues such as skin and bone have a high electrical resistance that makes it difficult, if not impossible, to stimulate the underlying nerves either non-invasively or painlessly- see Tissue electrical conductivity table. Needle electrodes can be used to overcome the barrier produced by the skin, but do not help with stimulating through bony structures such as the skull.
low frequency magnetic fields (tens of kHz and below) are able to pass unhindered through all biological tissue
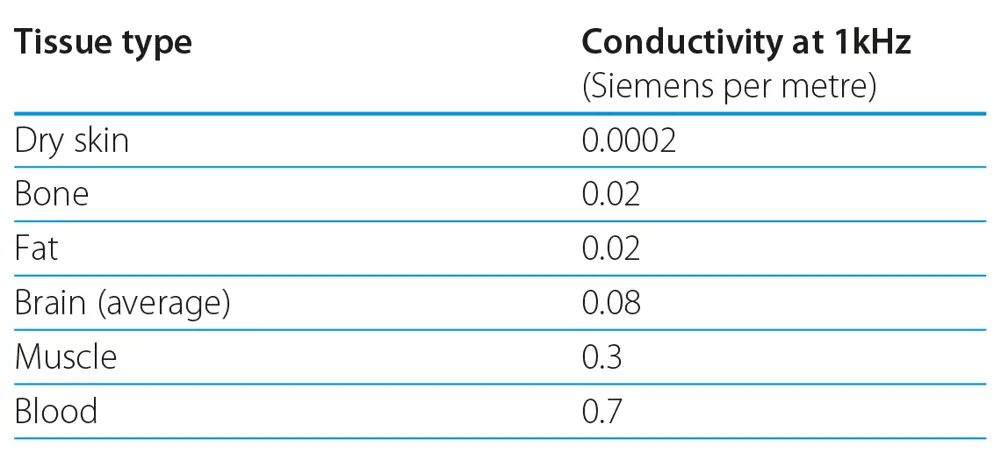
Tissue electrical conductivity table. Blood is a relatively good conductor and current flows readily trough it. Bone and fat are 30 times more resistant to current flow and dry skin is much more resistant making it very hard to pass current through it.
By contrast, low frequency magnetic fields (tens of kHz and below) are able to pass unhindered through all biological tissue. This led Barker to consider using magnetically induced currents to achieve the required stimulation. At these frequencies, brief but intense magnetic fields could induce electrical currents in human tissue, and so stimulate underlying nerves without causing pain and without having to expose the nerve or make any physical contact with it. By 1982, after seven years of research, Barker and colleagues had devised the first functional magnetic stimulator.
Elsewhere, in 1980, the neuroscientists PA Merton and HB Morton had demonstrated that it was possible to stimulate the motor area of the human cortex by applying electrodes to the scalp and administering brief, high-voltage electric shocks. Although a potentially valuable technique in brain research, its use was limited because the shocks also activated pain receptors in the subjects’ scalps, causing considerable discomfort. On hearing of their work, Barker contacted Merton and arranged to demonstrate the progress made with the Sheffield magnetic stimulator in his laboratory at the National Hospital for Nervous Diseases in London. On 12 February 1985, the stimulating coil was placed on Merton’s head, the stimulator was fired and his hand moved in what was the first demonstration of painless non–invasive brain stimulation. The technique of transcranial magnetic stimulation (TMS) was born.
[TMS] has become a research tool in many fields of neuroscience research and is becoming established in several therapeutic areas
In 1986, the Sheffield researchers licensed the technology to an American company, Novametrix Medical Systems Inc. Its UK subsidiary developed the Magstim 200, the first commercially available device. In 1990, a syndicate of Novametrix’s UK managers purchased the rights to the device and set up what is now the Magstim Company Ltd, which remains a major manufacturer of magnetic stimulation equipment based in Whitland, Wales, with a workforce of 100 people. They produce equipment that can be used for research, diagnosis, prognosis and therapy in a range of nervous and psychiatric disorders.
Now, TMS is used to excite or inhibit the brain, to make functional maps of the various regions of the cortex, that allow the function of those regions to be studied. Not surprisingly, it has become a research tool in many fields of neuroscience research and is becoming established in several therapeutic areas.
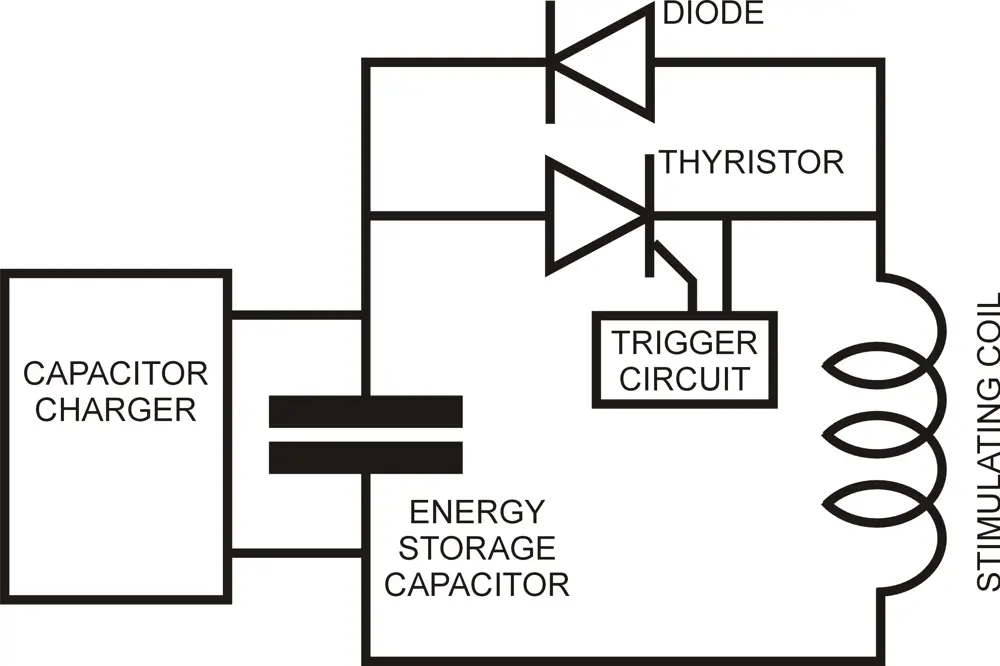
In this basic form of stimulator, the capacitor is charged, usually to several thousand volts and several hundred joules of energy. When the thyristor is triggered, this energy is discharged into the coil, creating a large magnetic field pulse
How TMS works
All cells in the body are surrounded by a membrane responsible for maintaining a difference in electrical potential between their interior and their surroundings. In a nerve cell, this potential difference is around -70 mV. A locally induced current, able to decrease this potential difference by a few millivolts somewhere along the length of the cell membrane, will initiate a wave of depolarisation that actively propagates along the length of the nerve fibre. This ‘action potential’ is what is commonly referred to as a nerve impulse.
An action potential can also be induced by inserting an electrode into, or near the nerve and stimulating it electrically, or by injecting current through the skin. The former is, of course, invasive and the latter causes discomfort by stimulating pain receptors in the skin. Faraday’s discovery pointed to a way of achieving the same end noninvasively and painlessly by inducing a current using an externally applied magnetic field.
Less straightforward is the actual engineering needed to generate the required fields. The challenges stem largely from the size of the parameters involved
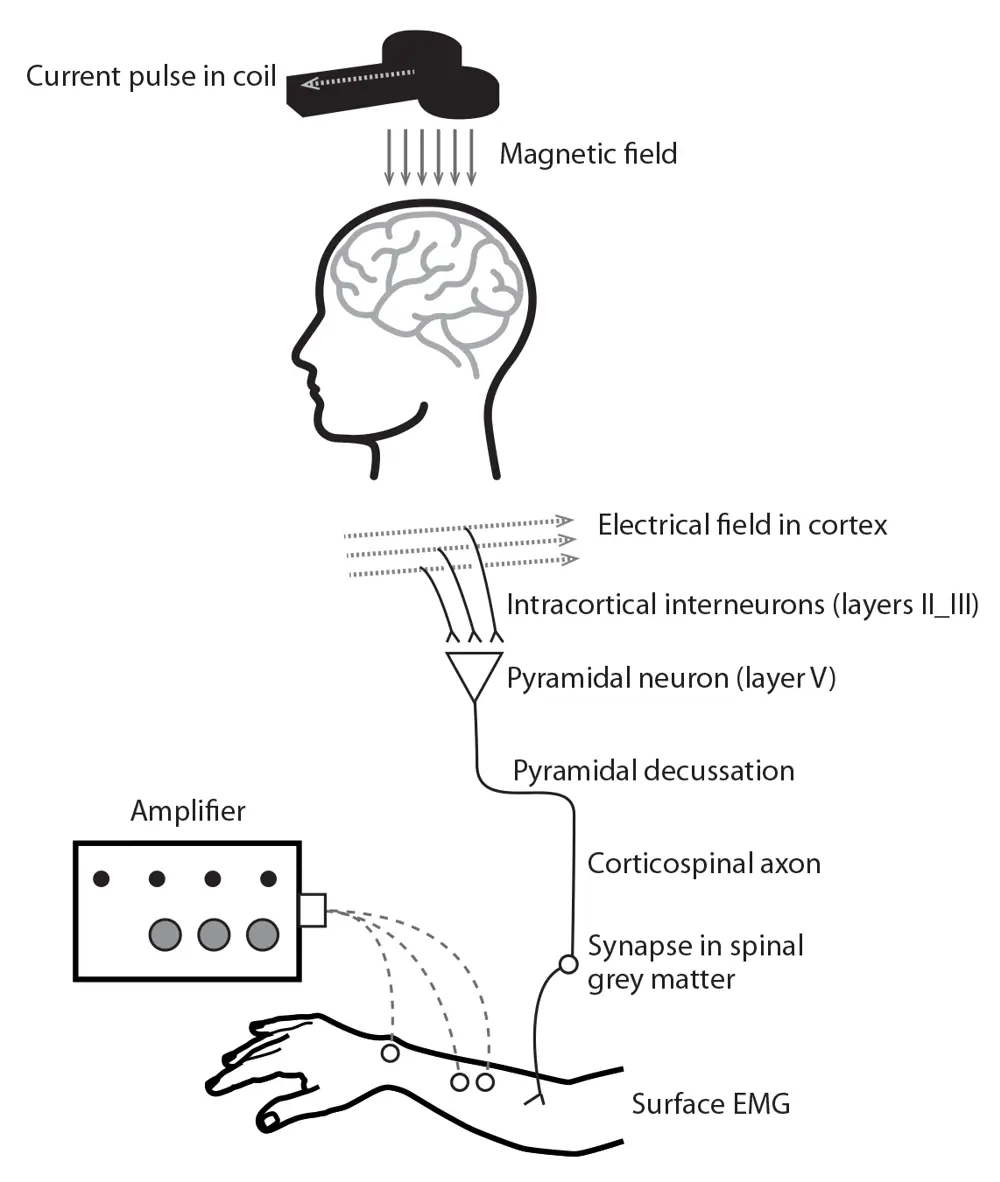
Schematic showing the complete pathway of magnetic stimulation, from coil to muscle response
The equipment required to generate suitable magnetic field pulses comprises a power source to charge a single, large capacitor that feeds a coil placed on the surface of the body above the nerves to be stimulated. Discharging the capacitor into the coil generates a pulse of magnetic field, which in turn sets up a current in the nerve, and initiates a nerve impulse. The original stimulators delivered single pulses, with a maximum repetition rate of about one pulse every five seconds. Today’s therapy devices can deliver up to 30 or more pulses per second and have a more complex circuit topography to enable energy from the initial pulse to be partly recaptured, decreasing the additional energy required to deliver the next pulse.
Less straightforward is the actual engineering needed to generate the required fields. The challenges stem largely from the size of the parameters involved. Depending on the coil type, peak magnetic fields of up to 4 Tesla are needed to induce the necessary currents in the tissue and this, in turn, requires peak currents in the coil of several thousand amps. In order to couple efficiently with the nerve membrane, a capacitor voltage of several thousand volts is needed to overcome the stimulating coil inductance and achieve a fast field risetime of around 100 μs.
Building the capacitors and coils
Most coils are built in the shape of a circle or a figure of eight. The typical circular coil comprises nine or so turns of thick enamelled copper wire, rectangular in cross section and induces current loops in the tissue that are approximately circular. Each time the system is fired, the windings attract one another. The forces involved can be of the order of 10,000 Newtons, which leads to relative movement between the windings, and to the clicking sound emitted by some instruments. One way of mitigating the effects of these movements is to cushion the winding. This can be done by using a thin layer of hot melt adhesive, a thermoplastic glue applied to the wires during the winding process.
The figure-of-eight configuration comprises two circular coils placed edge to edge and wired so that the currents in them rotate in opposite directions. Each coil induces circular current loops in the tissue but in opposite directions. The principle of superposition applies, making the induced currents in the centre of the configuration approximately twice those that occur elsewhere. This gives the figure of eight coil a degree of spatial ‘focality’, with stimulation most likely to occur at this midpoint.
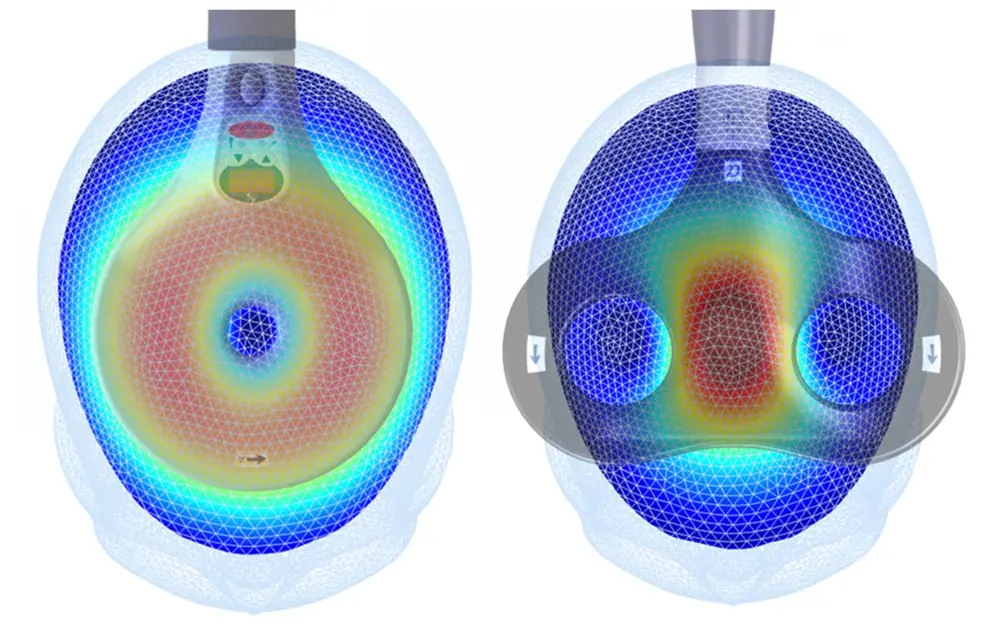
This picture shows the patterns of induced electric field in a simple model of the head from a circular (left) and a figure of eight coil (right). The strength of the induced field is represented by colour: black being zero; cyan modest fields; red strong fields and dark red the maximum © Magstim
Coils can be made in different sizes. The smaller the coil, the greater the spatial precision of the stimulus – but the fall off in magnetic field strength is more rapid, and so also is the induced current strength, with distance from the coil. A big coil will stimulate deeper structures but with poor spatial selectivity; a small coil will stimulate only superficial structures, but with a better focus.
A typical figure of eight coil used clinically, with winding mean diameters of 70 mm will have a range of approximately 30 x 20 mm where the induced field is high, and it is in this region that stimulation is most likely. This high field area exists from just under the coil, where it is greatest, to several centimetres down into the tissue, and there is uncertainty as to the exact site of stimulation in all three dimensions. Computer modelling is now being used extensively to aid in the evaluation of the delivered stimulus.
By choosing the voltage to which the capacitors are charged, the operator can vary the peak magnetic field generated by the stimulator
The current induced in tissue lying along the central axis of a circular coil is zero. Maximum stimulation occurs beneath the winding. When stimulating, say, a peripheral nerve in the arm, the operator places a circular coil on the skin in such a way that the nerve beneath aligns tangentially with the mean diameter of the overlying coil. The maximum induced current will then flow along its length, so giving the greatest likelihood of stimulation.
By choosing the voltage to which the capacitors are charged, the operator can vary the peak magnetic field generated by the stimulator. This gives control over the strength of the delivered stimulus and affects the number of nerves being stimulated, and also allows their excitability (that is, their sensitivity to being stimulated) to be assessed.
Continuing research
🧠 New coil designs to deposit energy safely into deeper parts of the brain
The need for deeper but safe stimulation has prompted research into new coil designs. One approach being investigated by a team led by Professor David Jiles FREng, first at Cardiff University then Iowa State University in the US, is termed the halo. The concept of the halo is to use a coil with a diameter large enough to encircle the patient’s whole head, used in conjunction with a smaller, conventional coil. The aim is to increase the effective depth of penetration of the stimulus by allowing more energy to be deposited into deeper parts of the brain.
The large coil aims to provide much of the magnetic flux density, but at a level insufficient to achieve stimulation. The smaller coil would then be used to lift the field above the stimulation threshold in the region of interest. Work so far has been limited to computer models and laboratory simulations. One study measured the magnitude of the magnetic field at various distances below a circular coil without and with a halo coil. The latter was found to boost the magnetic field by 10% at a distance of 20 mm below the circular coil, and 50% at 50 mm.
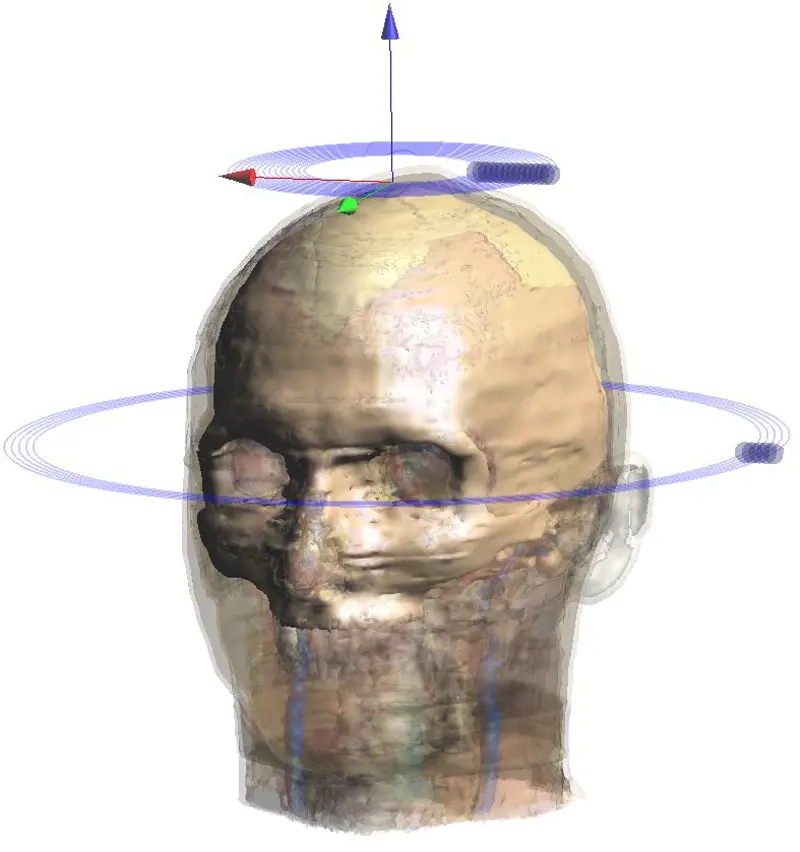
The larger ‘halo’ coil generates a sub-threshold field at depth which augments the magnetic field of the smaller coil, enabling it to stimulate at greater depths
In the lab and clinic
Magnetic stimulation, especially of specific regions of the brain, has generated a large number of research studies, adding greatly to the understanding of normal brain function. Following the single paper by the Sheffield group in 1985 describing their successful demonstration of TMS, over 10,000 papers on the topic have been published since. Although the clinical use of TMS is comparatively limited, it is these applications that understandably garner the most intense interest.
Regular monitoring can contribute to patients’ quality of life by alerting their doctors to an impending relapse, and permitting a more timely intervention
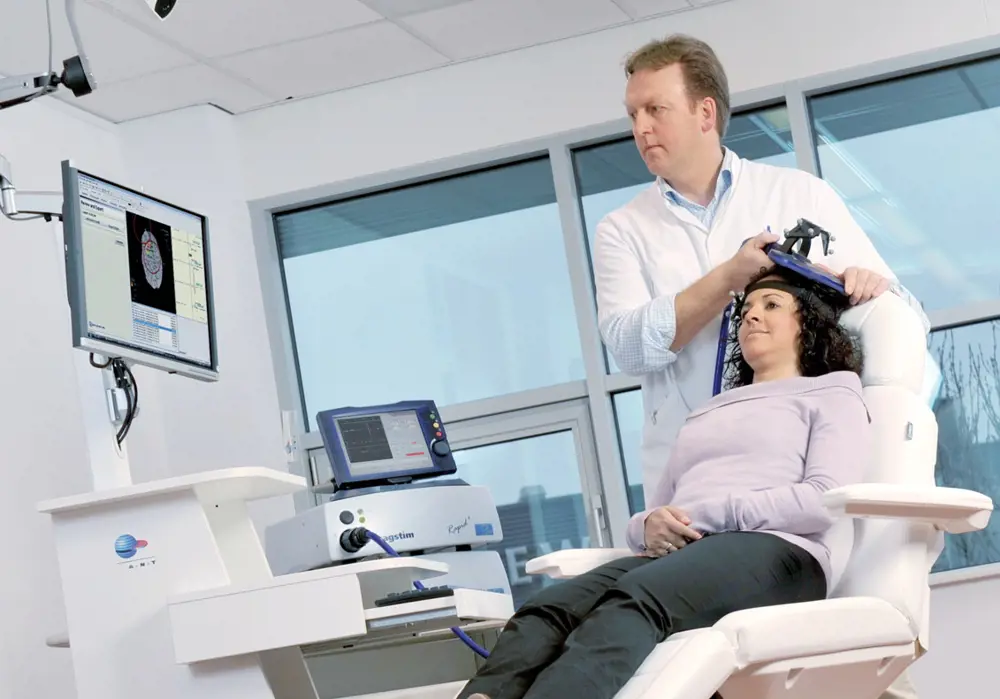
A magnetic stimulator coil is positioned on the head using a neuronavigation system which displays the actual coil location on an MRI image of the subject’s brain © Magstim
One of the most firmly established is in monitoring the conduction velocity of motor nerves in conditions, notably multiple sclerosis, where this is compromised. Magnetic stimulation allows conduction speeds to be quantified, and the underlying physiological failures to be identified and characterised. Regular monitoring can contribute to patients’ quality of life by alerting their doctors to an impending relapse, and permitting a more timely intervention.
The best evidence of therapeutic benefit comes from using TMS to treat depression and migraine. TMS has been shown to either excite or suppress activity in the brain, depending on the frequency with which the stimuli are delivered and this can be used to beneficial effect. In comparison with the best known non-drug physical treatment for severe depression, electro-convulsive therapy (ECT), TMS is a benign therapy with virtually no side effects and which does not need the use of anaesthesia.
Although TMS was approved as a treatment for medication-resistant depression in Canada in 2002, the UK government’s National Institute for Health and Clinical excellence (NICE) has only recently issued guidance on its approved use. A set of guidance recommendations, published in December 2015 states that magnetic stimulation raises no major safety concerns, and adds that “The evidence on its efficacy in the short‑term is adequate, although the clinical response is variable. Repetitive transcranial magnetic stimulation for depression may be used with normal arrangements for clinical governance and audit.”
[in treating depression and migraine] TMS is a benign therapy with virtually no side effects and which does not need the use of anaesthesia
An even more recent application of TMS is in suppressing the symptoms of migraine. The treatment can be self-administered at home or work when needed, using a battery-powered portable stimulator the size of a small briefcase. In guidance published at the beginning of 2014, NICE offered qualified approval. While pointing out that some of the evidence remains contradictory, it suggested that the technique can be used in two ways: to treat or reduce the severity of the migraine when it starts; or, by regular use, to prevent or reduce the frequency of migraines occurring. It added that “TMS treatment may have a particular role in the reduction or avoidance of drug therapy, either where preferred or where contraindicated or best avoided (for example, in pregnancy).”
The number of disorders that can claim at least one study of the benefits of magnetic stimulation – many more in some cases – is already lengthy and still growing. As identified in a report on novel neurotechnologies issued by the Nuffield Council on Bioethics, the list includes schizophrenia, Tourette’s syndrome, pain, addiction, epilepsy, memory loss, anxiety disorders, tinnitus, obsessive compulsive disorders, sleep, movement disorders (dystonia, Parkinson’s disease, ataxia) and stroke.
Some of these applications may fall by the wayside as further studies are completed; however, depression and migraine look set to become well-established applications and many others are being actively studied at present. It seems reasonable to expect that TMS will become an increasingly valuable therapeutic tool over the coming years.
***
This article has been adapted from "Transcranial magnetic stimulation", which originally appeared in the print edition of Ingenia 66 (March 2016).
Contributors
Geoff Watts
Author
Mike Polson has been active in the development of electromedical devices since receiving his PhD at Sheffield in 1982. He was Engineering Director at Magstim between 2013 and 2019 and is now the Proprietor of DyMed Consulting.
Keep up-to-date with Ingenia for free
SubscribeRelated content
Health & medical
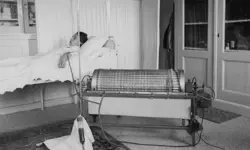
Kidney dialysis
Small haemodialysis machines have been developed that will allow more people to treat themselves at home. The SC+ system that has been developed is lighter, smaller and easier to use than existing machines.
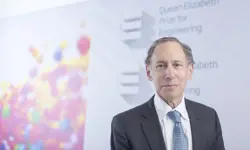
Engineering polymath wins major award
The 2015 Queen Elizabeth Prize for Engineering has been awarded to the ground-breaking chemical engineer Dr Robert Langer FREng for his revolutionary advances and leadership in engineering at the interface between chemistry and medicine.
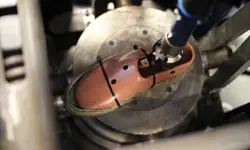
Blast mitigation and injury treatment
The Royal British Legion Centre for Blast Injury Studies is a world-renowned research facility based at Imperial College London. Its director, Professor Anthony Bull FREng, explains how a multidisciplinary team is helping protect, treat and rehabilitate people who are exposed to explosive forces.
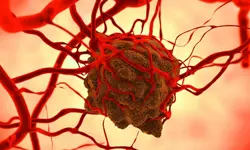
Targeting cancers with magnetism
Cambridge-based Endomag has helped treat more than 6,000 breast cancer patients across 20 countries. The MacRobert finalist uses magnetic fields to power diagnostic and therapeutic devices. Find about the challenges that surround the development and acceptance of medical innovations.
Other content from Ingenia
Quick read

- Environment & sustainability
- Opinion
A young engineer’s perspective on the good, the bad and the ugly of COP27

- Environment & sustainability
- Issue 95
How do we pay for net zero technologies?
Quick read

- Transport
- Mechanical
- How I got here
Electrifying trains and STEMAZING outreach

- Civil & structural
- Environment & sustainability
- Issue 95